17
The Laws of Induction
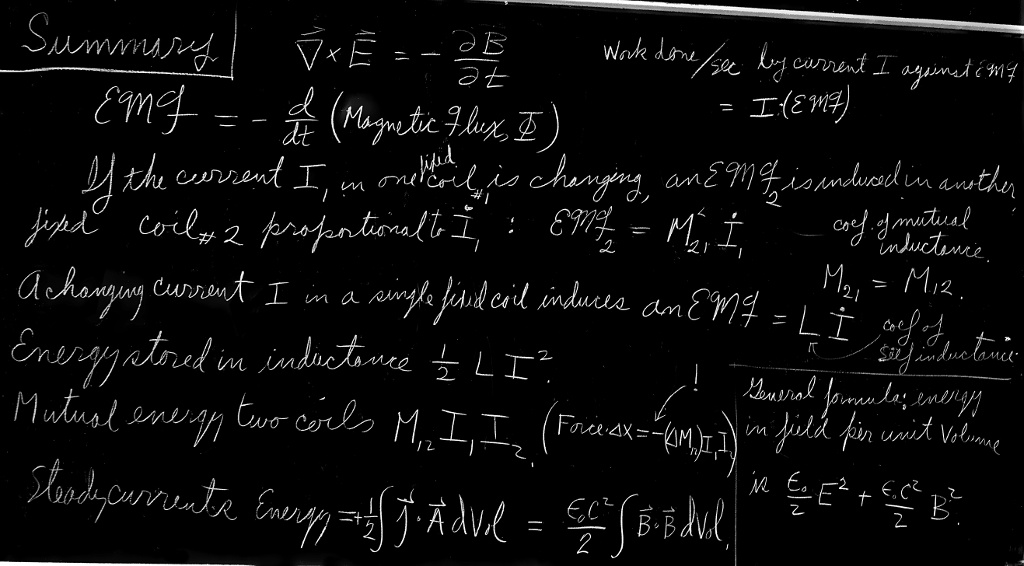
17–1The physics of induction
In the last chapter we described many phenomena which show that the effects of induction are quite complicated and interesting. Now we want to discuss the fundamental principles which govern these effects. We have already defined the emf in a conducting circuit as the total accumulated force on the charges throughout the length of the loop. More specifically, it is the tangential component of the force per unit charge, integrated along the wire once around the circuit. This quantity is equal, therefore, to the total work done on a single charge that travels once around the circuit.
We have also given the “flux rule,” which says that the emf is equal to the rate at which the magnetic flux through such a conducting circuit is changing. Let’s see if we can understand why that might be. First, we’ll consider a case in which the flux changes because a circuit is moved in a steady field.
In Fig. 17–1 we show a simple loop of wire whose dimensions can be changed. The loop has two parts, a fixed U-shaped part (a) and a movable crossbar (b) that can slide along the two legs of the U. There is always a complete circuit, but its area is variable. Suppose we now place the loop in a uniform magnetic field with the plane of the U perpendicular to the field. According to the rule, when the crossbar is moved there should be in the loop an emf that is proportional to the rate of change of the flux through the loop. This emf will cause a current in the loop. We will assume that there is enough resistance in the wire that the currents are small. Then we can neglect any magnetic field from this current.
The flux through the loop is $wLB$, so the “flux rule” would give for the emf—which we write as $\emf$— \begin{equation*} \emf=wB\,\ddt{L}{t}=wBv, \end{equation*} where $v$ is the speed of translation of the crossbar.
Now we should be able to understand this result from the magnetic $\FLPv\times\FLPB$ forces on the charges in the moving crossbar. These charges will feel a force, tangential to the wire, equal to $vB$ per unit charge. It is constant along the length $w$ of the crossbar and zero elsewhere, so the integral is \begin{equation*} \emf=wvB, \end{equation*} which is the same result we got from the rate of change of the flux.
The argument just given can be extended to any case where there is a fixed magnetic field and the wires are moved. One can prove, in general, that for any circuit whose parts move in a fixed magnetic field the emf is the time derivative of the flux, regardless of the shape of the circuit.
2024.7.10: To see this, 위 논리를 잘 들여다 보면
1. 각 도선 element $ds$를 넘나드는 넓이 변화 문제고
2. 그건 $\FLPv \times \FLPB \cdot {ds}$니 그걸 전부 합한, 즉 integral
$\int \FLPv\times\FLPB \cdot d\FLPs $가 flux 변화율
On the other hand, what happens if the loop is stationary and the magnetic field is changed? We cannot deduce the answer to this question from the same argument. It was Faraday’s discovery—from experiment—that the “flux rule” is still correct no matter why the flux changes. The force on electric charges is given in complete generality by $\FLPF=q(\FLPE+\FLPv\times\FLPB)$; there are no new special “forces due to changing magnetic fields.” Any forces on charges at rest in a stationary wire come from the $\FLPE$ term. Faraday’s observations led to the discovery that electric and magnetic fields are related by a new law: in a region where the magnetic field is changing with time, electric fields are generated. It is this electric field which drives the electrons around the wire—and so is responsible for the emf in a stationary circuit when there is a changing magnetic flux.
The general law for the electric field associated with a changing magnetic field is \begin{equation} \label{Eq:II:17:1} \FLPcurl{\FLPE}=-\ddp{\FLPB}{t}. \end{equation} We will call this Faraday’s law. It was discovered by Faraday but was first written in differential form by Maxwell, as one of his equations. Let’s see how this equation gives the “flux rule” for circuits.
Using Stokes’ theorem, this law can be written in integral form as \begin{equation} \label{Eq:II:17:2} \oint_\Gamma\FLPE\cdot d\FLPs= \int_S(\FLPcurl{\FLPE})\cdot\FLPn\,da= -\int_S\ddp{\FLPB}{t}\cdot\FLPn\,da, \end{equation} where, as usual, $\Gamma$ is any closed curve and $S$ is any surface bounded by it. Here, remember, $\Gamma$ is a mathematical curve fixed in space, and $S$ is a fixed surface. Then the time derivative can be taken outside the integral and we have \begin{equation} \begin{aligned} \oint_\Gamma\FLPE\cdot d\FLPs& =-\ddt{}{t}\int_S\FLPB\cdot\FLPn\,da \\[1ex] &=-\ddt{}{t}(\text{flux through $S$}). \end{aligned} \label{Eq:II:17:3} \end{equation} Applying this relation to a curve $\Gamma$ that follows a fixed circuit of conductor, we get the “flux rule” once again. The integral on the left is the emf, and that on the right is the negative rate of change of the flux linked by the circuit. So Eq. (17.1) applied to a fixed circuit is equivalent to the “flux rule.”
So the “flux rule”—that the emf in a circuit is equal to the rate of change of the magnetic flux through the circuit—applies whether the flux changes because the field changes or because the circuit moves (or both). The two possibilities—“circuit moves” or “field changes”—are not distinguished in the statement of the rule. Yet in our explanation of the rule we have used two completely distinct laws for the two cases—$\FLPv\times\FLPB$ for “circuit moves” and $\FLPcurl{\FLPE}=-\ddpl{\FLPB}{t}$ for “field changes.”
We know of no other place in physics where such a simple and accurate general principle requires for its real understanding an analysis in terms of two different phenomena. Usually such a beautiful generalization is found to stem from a single deep underlying principle. Nevertheless, in this case there does not appear to be any such profound implication. We have to understand the “rule” as the combined effects of two quite separate phenomena.
We must look at the “flux rule” in the following way. In general, the force per unit charge is $\FLPF/q=\FLPE+\FLPv\times\FLPB$. In moving wires there is the force from the second term. Also, there is an $\FLPE$-field if there is somewhere a changing magnetic field. They are independent effects, but the emf around the loop of wire is always equal to the rate of change of magnetic flux through it.
17–2Exceptions to the “flux rule”
We will now give some examples, due in part to Faraday, which show the importance of keeping clearly in mind the distinction between the two effects responsible for induced emf’s. Our examples involve situations to which the “flux rule” cannot be applied—either because there is no wire at all or because the path taken by induced currents moves about within an extended volume of a conductor.
We begin by making an important point: The part of the emf that comes from the $\FLPE$-field does not depend on the existence of a physical wire (as does the $\FLPv\times\FLPB$ part). The $\FLPE$-field can exist in free space, and its line integral around any imaginary line fixed in space is the rate of change of the flux of $\FLPB$ through that line. (Note that this is quite unlike the $\FLPE$-field produced by static charges, for in that case the line integral of $\FLPE$ around a closed loop is always zero.)
Now we will describe a situation in which the flux through a circuit does not change, but there is nevertheless an emf. Figure 17–2 shows a conducting disc which can be rotated on a fixed axis in the presence of a magnetic field. One contact is made to the shaft and another rubs on the outer periphery of the disc. A circuit is completed through a galvanometer. As the disc rotates, the “circuit,” in the sense of the place in space where the currents are, is always the same. But the part of the “circuit” in the disc is in material which is moving. Although the flux through the “circuit” is constant, there is still an emf, as can be observed by the deflection of the galvanometer. Clearly, here is a case where the $\FLPv\times\FLPB$ force in the moving disc gives rise to an emf which cannot be equated to a change of flux.
Now we consider, as an opposite example, a somewhat unusual situation in which the flux through a “circuit” (again in the sense of the place where the current is) changes but where there is no emf. Imagine two metal plates with slightly curved edges, as shown in Fig. 17–3, placed in a uniform magnetic field perpendicular to their surfaces. Each plate is connected to one of the terminals of a galvanometer, as shown. The plates make contact at one point $P$, so there is a complete circuit. If the plates are now rocked through a small angle, the point of contact will move to $P'$. If we imagine the “circuit” to be completed through the plates on the dotted line shown in the figure, the magnetic flux through this circuit changes by a large amount as the plates are rocked back and forth. Yet the rocking can be done with small motions, so that $\FLPv\times\FLPB$ is very small and there is practically no emf. The “flux rule” does not work in this case. It must be applied to circuits in which the material of the circuit remains the same. When the material of the circuit is changing, we must return to the basic laws. The correct physics is always given by the two basic laws \begin{align*} &\FLPF=q(\FLPE+\FLPv\times\FLPB),\\[1ex] &\FLPcurl{\FLPE}=-\ddp{\FLPB}{t}. \end{align*}
17–3Particle acceleration by an induced electric field; the betatron
We have said that the electromotive force generated by a changing magnetic field can exist even without conductors; that is, there can be magnetic induction without wires. We may still imagine an electromotive force around an arbitrary mathematical curve in space. It is defined as the tangential component of $\FLPE$ integrated around the curve. Faraday’s law says that this line integral is equal to minus the rate of change of the magnetic flux through the closed curve, Eq. (17.3).
As an example of the effect of such an induced electric field, we want now to consider the motion of an electron in a changing magnetic field. We imagine a magnetic field which, everywhere on a plane, points in a vertical direction, as shown in Fig. 17–4. The magnetic field is produced by an electromagnet, but we will not worry about the details. For our example we will imagine that the magnetic field is symmetric about some axis, i.e., that the strength of the magnetic field will depend only on the distance from the axis. The magnetic field is also varying with time. We now imagine an electron that is moving in this field on a path that is a circle of constant radius with its center at the axis of the field. (We will see later how this motion can be arranged.) Because of the changing magnetic field, there will be an electric field $\FLPE$ tangential to the electron’s orbit which will drive it around the circle. Because of the symmetry, this electric field will have the same value everywhere on the circle. If the electron’s orbit has the radius $r$, the line integral of $\FLPE$ around the orbit is equal to minus the rate of change of the magnetic flux through the circle. The line integral of $\FLPE$ is just its magnitude times the circumference of the circle, $2\pi r$. The magnetic flux must, in general, be obtained from an integral. For the moment, we let $B_{\text{av}}$ represent the average magnetic field in the interior of the circle; then the flux is this average magnetic field times the area of the circle. We will have \begin{equation*} 2\pi rE=\ddt{}{t}(B_{\text{av}}\cdot\pi r^2). \end{equation*}
Since we are assuming $r$ is constant, $E$ is proportional to the time derivative of the average field: \begin{equation} \label{Eq:II:17:4} E=\frac{r}{2}\,\ddt{B_{\text{av}}}{t}. \end{equation} The electron will feel the electric force $q\FLPE$ and will be accelerated by it. Remembering that the relativistically correct equation of motion is that the rate of change of the momentum is proportional to the force, we have \begin{equation} \label{Eq:II:17:5} qE=\ddt{p}{t}. \end{equation}
For the circular orbit we have assumed, the electric force on the electron is always in the direction of its motion, so its total momentum will be increasing at the rate given by Eq. (17.5). Combining Eqs. (17.5) and (17.4), we may relate the rate of change of momentum to the change of the average magnetic field: \begin{equation} \label{Eq:II:17:6} \ddt{p}{t}=\frac{qr}{2}\,\ddt{B_{\text{av}}}{t}. \end{equation} Integrating with respect to $t$, we find for the electron’s momentum \begin{equation} \label{Eq:II:17:7} p=p_0+\frac{qr}{2}\,\Delta B_{\text{av}}, \end{equation} where $p_0$ is the momentum with which the electrons start out, and $\Delta B_{\text{av}}$, is the subsequent change in $B_{\text{av}}$. The operation of a betatron—a machine for accelerating electrons to high energies—is based on this idea.
To see how the betatron operates in detail, we must now examine how the electron can be constrained to move on a circle. We have discussed in Chapter 11 of Vol. I the principle involved. If we arrange that there is a magnetic field $\FLPB$ at the orbit of the electron, there will be a transverse force $q\FLPv\times\FLPB$ which, for a suitably chosen $\FLPB$, can cause the electron to keep moving on its assumed orbit. In the betatron this transverse force causes the electron to move in a circular orbit of constant radius. We can find out what the magnetic field at the orbit must be by using again the relativistic equation of motion, but this time, for the transverse component of the force. In the betatron (see Fig. 17–4), $\FLPB$ is at right angles to $\FLPv$, so the transverse force is $qvB$. Thus the force is equal to the rate of change of the transverse component $p_t$ of the momentum: \begin{equation} \label{Eq:II:17:8} qvB=\ddt{p_t}{t}. \end{equation} When a particle is moving in a circle, the rate of change of its transverse momentum is equal to the magnitude of the total momentum times $\omega$, the angular velocity of rotation (following the arguments of Chapter 11, Vol. I): \begin{equation} \label{Eq:II:17:9} \ddt{p_t}{t}=\omega p, \end{equation} where, since the motion is circular, \begin{equation} \label{Eq:II:17:10} \omega=\frac{v}{r}. \end{equation} Setting the magnetic force equal to the transverse acceleration, we have \begin{equation} \label{Eq:II:17:11} qvB_{\text{orbit}}=p\,\frac{v}{r}, \end{equation} where $B_{\text{orbit}}$ is the field at the radius $r$.
As the betatron operates, the momentum of the electron grows in proportion to $B_{\text{av}}$, according to Eq. (17.7), and if the electron is to continue to move in its proper circle, Eq. (17.11) must continue to hold as the momentum of the electron increases. The value of $B_{\text{orbit}}$ must increase in proportion to the momentum $p$. Comparing Eq. (17.11) with Eq. (17.7), which determines $p$, we see that the following relation must hold between $B_{\text{av}}$, the average magnetic field inside the orbit at the radius $r$, and the magnetic field $B_{\text{orbit}}$ at the orbit: \begin{equation} \label{Eq:II:17:12} \Delta B_{\text{av}}=2\,\Delta B_{\text{orbit}}. \end{equation} The correct operation of a betatron requires that the average magnetic field inside the orbit increases at twice the rate of the magnetic field at the orbit itself. In these circumstances, as the energy of the particle is increased by the induced electric field the magnetic field at the orbit increases at just the rate required to keep the particle moving in a circle.
The betatron is used to accelerate electrons to energies of tens of millions of volts, or even to hundreds of millions of volts. However, it becomes impractical for the acceleration of electrons to energies much higher than a few hundred million volts for several reasons. One of them is the practical difficulty of attaining the required high average value for the magnetic field inside the orbit. Another is that Eq. (17.6) is no longer correct at very high energies because it does not include the loss of energy from the particle due to its radiation of electromagnetic energy (the so-called synchrotron radiation discussed in Chapter 34, Vol. I). For these reasons, the acceleration of electrons to the highest energies—to many billions of electron volts—is accomplished by means of a different kind of machine, called a synchrotron.
17–4A paradox
We would now like to describe for you an apparent paradox. A paradox is a situation which gives one answer when analyzed one way, and a different answer when analyzed another way, so that we are left in somewhat of a quandary as to actually what should happen. Of course, in physics there are never any real paradoxes because there is only one correct answer; at least we believe that nature will act in only one way (and that is the right way, naturally). So in physics a paradox is only a confusion in our own understanding. Here is our paradox.
Imagine that we construct a device like that shown in Fig. 17–5. There is a thin, circular plastic disc supported on a concentric shaft with excellent bearings, so that it is quite free to rotate. On the disc is a coil of wire in the form of a short solenoid concentric with the axis of rotation. This solenoid carries a steady current $I$ provided by a small battery, also mounted on the disc. Near the edge of the disc and spaced uniformly around its circumference are a number of small metal spheres insulated from each other and from the solenoid by the plastic material of the disc. Each of these small conducting spheres is charged with the same electrostatic charge $Q$. Everything is quite stationary, and the disc is at rest. Suppose now that by some accident—or by prearrangement—the current in the solenoid is interrupted, without, however, any intervention from the outside. So long as the current continued, there was a magnetic flux through the solenoid more or less parallel to the axis of the disc. When the current is interrupted, this flux must go to zero. There will, therefore, be an electric field induced which will circulate around in circles centered at the axis. The charged spheres on the perimeter of the disc will all experience an electric field tangential to the perimeter of the disc. This electric force is in the same sense for all the charges and so will result in a net torque on the disc. From these arguments we would expect that as the current in the solenoid disappears, the disc would begin to rotate. If we knew the moment of inertia of the disc, the current in the solenoid, and the charges on the small spheres, we could compute the resulting angular velocity.
But we could also make a different argument. Using the principle of the conservation of angular momentum, we could say that the angular momentum of the disc with all its equipment is initially zero, and so the angular momentum of the assembly should remain zero. There should be no rotation when the current is stopped. Which argument is correct? Will the disc rotate or will it not? We will leave this question for you to think about.
We should warn you that the correct answer does not depend on any nonessential feature, such as the asymmetric position of a battery, for example. In fact, you can imagine an ideal situation such as the following: The solenoid is made of superconducting wire through which there is a current. After the disc has been carefully placed at rest, the temperature of the solenoid is allowed to rise slowly. When the temperature of the wire reaches the transition temperature between superconductivity and normal conductivity, the current in the solenoid will be brought to zero by the resistance of the wire. The flux will, as before, fall to zero, and there will be an electric field around the axis. We should also warn you that the solution is not easy, nor is it a trick. When you figure it out, you will have discovered an important principle of electromagnetism.
17–5Alternating-current generator
In the remainder of this chapter we apply the principles of Section 17–1 to analyze a number of the phenomena discussed in Chapter 16. We first look in more detail at the alternating-current generator. Such a generator consists basically of a coil of wire rotating in a uniform magnetic field. The same result can also be achieved by a fixed coil in a magnetic field whose direction rotates in the manner described in the last chapter. We will consider only the former case. Suppose we have a circular coil of wire which can be turned on an axis along one of its diameters. Let this coil be located in a uniform magnetic field perpendicular to the axis of rotation, as in Fig. 17–6. We also imagine that the two ends of the coil are brought to external connections through some kind of sliding contacts.
Due to the rotation of the coil, the magnetic flux through it will be changing. The circuit of the coil will therefore have an emf in it. Let $S$ be the area of the coil and $\theta$ the angle between the magnetic field and the normal to the plane of the coil.1 The flux through the coil is then \begin{equation} \label{Eq:II:17:13} BS\cos\theta. \end{equation} If the coil is rotating at the uniform angular velocity $\omega$, $\theta$ varies with time as $\theta=\omega t$.
Each turn of the coil will have an emf equal to the rate of change of this flux. If the coil has $N$ turns of wire the total emf will be $N$ times larger, so \begin{equation} \label{Eq:II:17:14} \emf=-N\,\ddt{}{t}(BS\cos\omega t)=NBS\omega\sin\omega t. \end{equation}
If we bring the wires from the generator to a point some distance from the rotating coil, where the magnetic field is zero, or at least is not varying with time, the curl of $\FLPE$ in this region will be zero and we can define an electric potential. In fact, if there is no current being drawn from the generator, the potential difference $V$ between the two wires will be equal to the emf in the rotating coil. That is, \begin{equation*} V=NBS\omega\sin\omega t=V_0\sin\omega t. \end{equation*} The potential difference between the wires varies as $\sin\omega t$. Such a varying potential difference is called an alternating voltage.
Since there is an electric field between the wires, they must be electrically charged. It is clear that the emf of the generator has pushed some excess charges out to the wire until the electric field from them is strong enough to exactly counterbalance the induction force. Seen from outside the generator, the two wires appear as though they had been electrostatically charged to the potential difference $V$, and as though the charge was being changed with time to give an alternating potential difference. There is also another difference from an electrostatic situation. If we connect the generator to an external circuit that permits passage of a current, we find that the emf does not permit the wires to be discharged but continues to provide charge to the wires as current is drawn from them, attempting to keep the wires always at the same potential difference. If, in fact, the generator is connected in a circuit whose total resistance is $R$, the current through the circuit will be proportional to the emf of the generator and inversely proportional to $R$. Since the emf has a sinusoidal time variation, so also does the current. There is an alternating current \begin{equation*} I=\frac{\emf}{R}=\frac{V_0}{R}\sin\omega t. \end{equation*} The schematic diagram of such a circuit is shown in Fig. 17–7.
We can also see that the emf determines how much energy is supplied by the generator. Each charge in the wire is receiving energy at the rate $\FLPF\cdot\FLPv$, where $\FLPF$ is the force on the charge and $\FLPv$ is its velocity. Now let the number of moving charges per unit length of the wire be $n$; then the power being delivered into any element $ds$ of the wire is \begin{equation*} \FLPF\cdot\FLPv n\,ds. \end{equation*}
2024.7.13: light moment, pushing momentum
For a wire, $\FLPv$ is always along $d\FLPs$, so we can rewrite the power as \begin{equation*} nv\FLPF\cdot d\FLPs. \end{equation*} The total power being delivered to the complete circuit is the integral of this expression around the complete loop: \begin{equation} \label{Eq:II:17:15} \text{Power}=\oint nv\FLPF\cdot d\FLPs. \end{equation} Now remember that $qnv$ is the current $I$, and that the emf is defined as the integral of $F/q$ around the circuit. We get the result \begin{equation} \label{Eq:II:17:16} \text{Power from a generator}=\emf I. \end{equation}When there is a current in the coil of the generator, there will also be mechanical forces on it. In fact, we know that the torque on the coil is proportional to its magnetic moment, to the magnetic field strength $B$, and to the sine of the angle between. The magnetic moment is the current in the coil times its area. Therefore the torque is \begin{equation} \label{Eq:II:17:17} \tau=NISB\sin\theta. \end{equation} The rate at which mechanical work must be done to keep the coil rotating is the angular velocity $\omega$ times the torque: \begin{equation} \label{Eq:II:17:18} \ddt{W}{t}=\omega\tau=\omega NISB\sin\theta. \end{equation} Comparing this equation with Eq. (17.14), we see that the rate of mechanical work required to rotate the coil against the magnetic forces is just equal to $\emf I$, the rate at which electrical energy is delivered by the emf of the generator. All of the mechanical energy used up in the generator appears as electrical energy in the circuit.
As another example of the currents and forces due to an induced emf, let’s analyze what happens in the setup described in Section 17–1, and shown in Fig. 17–1. There are two parallel wires and a sliding crossbar located in a uniform magnetic field perpendicular to the plane of the parallel wires. Now let’s assume that the “bottom” of the U (the left side in the figure) is made of wires of high resistance, while the two side wires are made of a good conductor like copper—then we don’t need to worry about the change of the circuit resistance as the crossbar is moved. As before, the emf in the circuit is \begin{equation} \label{Eq:II:17:19} \emf=vBw. \end{equation} The current in the circuit is proportional to this emf and inversely proportional to the resistance of the circuit: \begin{equation} \label{Eq:II:17:20} I=\frac{\emf}{R}=\frac{vBw}{R}. \end{equation}
Because of this current there will be a magnetic force on the crossbar that is proportional to its length, to the current in it, and to the magnetic field, such that \begin{equation} \label{Eq:II:17:21} F=BIw. \end{equation} Taking $I$ from Eq. (17.20), we have for the force \begin{equation} \label{Eq:II:17:22} F=\frac{B^2w^2}{R}\,v. \end{equation} We see that the force is proportional to the velocity of the crossbar. The direction of the force, as you can easily see, is opposite to its velocity. Such a “velocity-proportional” force, which is like the force of viscosity, is found whenever induced currents are produced by moving conductors in a magnetic field. The examples of eddy currents we gave in the last chapter also produced forces on the conductors proportional to the velocity of the conductor, even though such situations, in general, give a complicated distribution of currents which is difficult to analyze.
It is often convenient in the design of mechanical systems to have damping forces which are proportional to the velocity. Eddy-current forces provide one of the most convenient ways of getting such a velocity-dependent force. An example of the application of such a force is found in the conventional domestic wattmeter. In the wattmeter there is a thin aluminum disc that rotates between the poles of a permanent magnet. This disc is driven by a small electric motor whose torque is proportional to the power being consumed in the electrical circuit of the house. Because of the eddy-current forces in the disc, there is a resistive force proportional to the velocity. In equilibrium, the velocity is therefore proportional to the rate of consumption of electrical energy. By means of a counter attached to the rotating disc, a record is kept of the number of revolutions it makes. This count is an indication of the total energy consumption, i.e., the number of watthours used.
We may also point out that Eq. (17.22) shows that the force from induced currents—that is, any eddy-current force—is inversely proportional to the resistance. The force will be larger, the better the conductivity of the material. The reason, of course, is that an emf produces more current if the resistance is low, and the stronger currents represent greater mechanical forces.
We can also see from our formulas how mechanical energy is converted into electrical energy. As before, the electrical energy supplied to the resistance of the circuit is the product $\emf I$. The rate at which work is done in moving the conducting crossbar is the force on the bar times its velocity. Using Eq. (17.22) for the force, the rate of doing work is \begin{equation*} \ddt{W}{t}=\frac{v^2B^2w^2}{R}. \end{equation*} We see that this is indeed equal to the product $\emf I$ we would get from Eqs. (17.19) and (17.20). Again the mechanical work appears as electrical energy.
17–6Mutual inductance
We now want to consider a situation in which there are fixed coils of wire but changing magnetic fields. When we described the production of magnetic fields by currents, we considered only the case of steady currents. But so long as the currents are changed slowly, the magnetic field will at each instant be nearly the same as the magnetic field of a steady current. We will assume in the discussion of this section that the currents are always varying sufficiently slowly that this is true.
In Fig. 17–8 is shown an arrangement of two coils which demonstrates the basic effects responsible for the operation of a transformer. Coil $1$ consists of a conducting wire wound in the form of a long solenoid. Around this coil—and insulated from it—is wound coil $2$, consisting of a few turns of wire. If now a current is passed through coil $1$, we know that a magnetic field will appear inside it. This magnetic field also passes through coil $2$. As the current in coil $1$ is varied, the magnetic flux will also vary, and there will be an induced emf in coil $2$. We will now calculate this induced emf.
We have seen in Section 13–5 that the magnetic field inside a long solenoid is uniform and has the magnitude \begin{equation} \label{Eq:II:17:23} B=\frac{1}{\epsO c^2}\,\frac{N_1I_1}{l}, \end{equation} where $N_1$ is the number of turns in coil $1$, $I_1$ is the current through it, and $l$ is its length. Let’s say that the cross-sectional area of coil $1$ is $S$; then the flux of $\FLPB$ is its magnitude times $S$. If coil $2$ has $N_2$ turns, this flux links the coil $N_2$ times. Therefore the emf in coil $2$ is given by \begin{equation} \label{Eq:II:17:24} \emf_2=-N_2S\,\ddt{B}{t}. \end{equation} The only quantity in Eq. (17.23) which varies with time is $I_1$. The emf is therefore given by \begin{equation} \label{Eq:II:17:25} \emf_2=-\frac{N_1N_2S}{\epsO c^2l}\,\ddt{I_1}{t}. \end{equation}
We see that the emf in coil $2$ is proportional to the rate of change of the current in coil $1$. The constant of proportionality, which is basically a geometric factor of the two coils, is called the mutual inductance, and is usually designated $\mutualInd_{21}$. Equation (17.25) is then written \begin{equation} \label{Eq:II:17:26} \emf_2=\mutualInd_{21}\,\ddt{I_1}{t}. \end{equation}
Suppose now that we were to pass a current through coil $2$ and ask about the emf in coil $1$. We would compute the magnetic field, which is everywhere proportional to the current $I_2$. The flux linkage through coil $1$ would depend on the geometry, but would be proportional to the current $I_2$. The emf in coil $1$ would, therefore, again be proportional to $dI_2/dt$: We can write \begin{equation} \label{Eq:II:17:27} \emf_1=\mutualInd_{12}\,\ddt{I_2}{t}. \end{equation} The computation of $\mutualInd_{12}$ would be more difficult than the computation we have just done for $\mutualInd_{21}$. We will not carry through that computation now, because we will show later in this chapter that $\mutualInd_{12}$ is necessarily equal to $\mutualInd_{21}$.
Since for any coil its field is proportional to its current, the same kind of result would be obtained for any two coils of wire. The equations (17.26) and (17.27) would have the same form; only the constants $\mutualInd_{21}$ and $\mutualInd_{12}$ would be different. Their values would depend on the shapes of the coils and their relative positions.
Suppose that we wish to find the mutual inductance between any two arbitrary coils—for example, those shown in Fig. 17–9. We know that the general expression for the emf in coil $1$ can be written as \begin{equation*} \emf_1=-\ddt{}{t}\int_{(1)}\FLPB\cdot\FLPn\,da, \end{equation*} where $\FLPB$ is the magnetic field and the integral is to be taken over a surface bounded by circuit $1$. We have seen in Section 14–1 that such a surface integral of $\FLPB$ can be related to a line integral of the vector potential. In particular, \begin{equation*} \int_{(1)}\FLPB\cdot\FLPn\,da=\oint_{(1)}\FLPA\cdot d\FLPs_1, \end{equation*} where $\FLPA$ represents the vector potential and $d\FLPs_1$ is an element of circuit $1$. The line integral is to be taken around circuit $1$. The emf in coil $1$ can therefore be written as \begin{equation} \label{Eq:II:17:28} \emf_1=-\ddt{}{t}\oint_{(1)}\FLPA\cdot d\FLPs_1. \end{equation}
Now let’s assume that the vector potential at circuit $1$ comes from currents in circuit $2$. Then it can be written as a line integral around circuit $2$: \begin{equation} \label{Eq:II:17:29} \FLPA=\frac{1}{4\pi\epsO c^2}\oint_{(2)}\frac{I_2\,d\FLPs_2}{r_{12}}, \end{equation} where $I_2$ is the current in circuit $2$, and $r_{12}$ is the distance from the element of the circuit $d\FLPs_2$ to the point on circuit $1$ at which we are evaluating the vector potential. (See Fig. 17–9.) Combining Eqs. (17.28) and (17.29), we can express the emf in circuit $1$ as a double line integral: \begin{equation*} \emf_1=-\frac{1}{4\pi\epsO c^2}\,\ddt{}{t}\oint_{(1)}\oint_{(2)} \frac{I_2\,d\FLPs_2}{r_{12}}\cdot d\FLPs_1. \end{equation*} In this equation the integrals are all taken with respect to stationary circuits. The only variable quantity is the current $I_2$, which does not depend on the variables of integration. We may therefore take it out of the integrals. The emf can then be written as \begin{equation*} \emf_1=\mutualInd_{12}\,\ddt{I_2}{t}, \end{equation*} where the coefficient $\mutualInd_{12}$ is \begin{equation} \label{Eq:II:17:30} \mutualInd_{12}=-\frac{1}{4\pi\epsO c^2}\oint_{(1)}\oint_{(2)} \frac{d\FLPs_2\cdot d\FLPs_1}{r_{12}}. \end{equation} We see from this integral that $\mutualInd_{12}$ depends only on the circuit geometry. It depends on a kind of average separation of the two circuits, with the average weighted most for parallel segments of the two coils.
* 셀프 인덕턴스 계산 => 토러스 solenoid
Our equation can be used for calculating the mutual inductance of any two circuits of arbitrary shape. Also, it shows that the integral for $\mutualInd_{12}$ is identical to the integral for $\mutualInd_{21}$. We have therefore shown that the two coefficients are identical. For a system with only two coils, the coefficients $\mutualInd_{12}$ and $\mutualInd_{21}$ are often represented by the symbol $\mutualInd$ without subscripts, called simply the mutual inductance: \begin{equation*} \mutualInd_{12}=\mutualInd_{21}=\mutualInd. \end{equation*}17–7Self-inductance
In discussing the induced electromotive forces in the two coils of Figs. 17–8 or 17–9, we have considered only the case in which there was a current in one coil or the other. If there are currents in the two coils simultaneously, the magnetic flux linking either coil will be the sum of the two fluxes which would exist separately, because the law of superposition applies for magnetic fields. The emf in either coil will therefore be proportional not only to the change of the current in the other coil, but also to the change in the current of the coil itself. Thus the total emf in coil $2$ should be written2 \begin{equation} \label{Eq:II:17:31} \emf_2=\mutualInd_{21}\,\ddt{I_1}{t}+\mutualInd_{22}\,\ddt{I_2}{t}. \end{equation} Similarly, the emf in coil $1$ will depend not only on the changing current in coil $2$, but also on the changing current in itself: \begin{equation} \label{Eq:II:17:32} \emf_1=\mutualInd_{12}\,\ddt{I_2}{t}+\mutualInd_{11}\,\ddt{I_1}{t}. \end{equation} The coefficients $\mutualInd_{22}$ and $\mutualInd_{11}$ are always negative numbers. It is usual to write \begin{equation} \label{Eq:II:17:33} \mutualInd_{11}=-\selfInd_1,\quad \mutualInd_{22}=-\selfInd_2, \end{equation} where $\selfInd_1$ and $\selfInd_2$ are called the self-inductances of the two coils.
The self-induced emf will, of course, exist even if we have only one coil. Any coil by itself will have a self-inductance $\selfInd$. The emf will be proportional to the rate of change of the current in it. For a single coil, it is usual to adopt the convention that the emf and the current are considered positive if they are in the same direction. With this convention, we may write for the emf of a single coil \begin{equation} \label{Eq:II:17:34} \emf=-\selfInd\,\ddt{I}{t}. \end{equation} The negative sign indicates that the emf opposes the change in current—it is often called a “back emf.”
Since any coil has a self-inductance which opposes the change in current, the current in the coil has a kind of inertia.
* current in a coil에 대한 생각(21.7.22)
electric field의 소용돌이로 볼 수 있고 electric field index에 변화를 주는데,
Poincaré-Hopf 정리에 의하여, 전체 인덱스 합은 일정하니 negative index에 해당되는 소용돌이가 생겨야 한다. 그것이 역류일 게다. => Lenz’s rule
인덕턴스가 geometry와 상관 즉 구조적이라는 것 => 토러스 인덕턴스
V와 emf의 차이: V는 포텐셜 에너지 차이, emf는 tangential force per unit charge 'in the wire' integrated over length, 즉 도선에서만 고려한다는 것 그리고 부호가 다르다는 것
참조: linear system
This equation has the same form as Newton’s law of motion for a particle in one dimension. We can therefore study it by the principle that “the same equations have the same solutions.” Thus, if we make the externally applied voltage $\voltage$ correspond to an externally applied force $F$, and the current $I$ in a coil correspond to the velocity $v$ of a particle, the inductance $\selfInd$ of the coil corresponds to the mass $m$ of the particle.3 See Fig. 17–10(b). We can make the following table of corresponding quantities(* 더 table) .
Particle | Coil |
$\begin{align*} &F\text{ (force)}\\[.5ex] &v\text{ (velocity)}\\[.5ex] &x\text{ (displacement)} \end{align*}$ | $\begin{align*} &\voltage\text{ (potential difference)}\\[.5ex] &I\text{ (current)}\\[.5ex] &q\text{ (charge)} \end{align*}$ |
$\displaystyle F=m\,\ddt{v}{t}$ | $\displaystyle \voltage=\selfInd\,\ddt{I}{t}$ |
$mv\text{ (momentum)}$ | $\selfInd I$ |
$\tfrac{1}{2}mv^2\text{ (kinetic energy)}$ | $\tfrac{1}{2}\selfInd I^2\text{ (magnetic energy)}$ |
17–8Inductance and magnetic energy
Continuing with the analogy of the preceding section, we would expect that corresponding to the mechanical momentum $p=mv$, whose rate of change is the applied force, there should be an analogous quantity equal to $\selfInd I$, whose rate of change is $\voltage$. We have no right, of course, to say that $\selfInd I$ is the real momentum of the circuit; in fact, it isn’t. The whole circuit may be standing still and have no momentum. It is only that $\selfInd I$ is analogous to the momentum $mv$ in the sense of satisfying corresponding equations. In the same way, to the kinetic energy $\tfrac{1}{2}mv^2$, there corresponds an analogous quantity $\tfrac{1}{2}\selfInd I^2$. But there we have a surprise. This $\tfrac{1}{2}\selfInd I^2$ is really the energy in the electrical case also. This is because the rate of doing work on the inductance is $\voltage I$, and in the mechanical system it is $Fv$, the corresponding quantity. Therefore, in the case of the energy, the quantities not only correspond mathematically, but also have the same physical meaning as well.
We may see this in more detail as follows. As we found in Eq. (17.16), the rate of electrical work by induced forces is the product of the electromotive force and the current: \begin{equation*} \ddt{W}{t}=\emf I. \end{equation*} Replacing $\emf$ by its expression in terms of the current from Eq. (17.34), we have \begin{equation} \label{Eq:II:17:36} \ddt{W}{t}=-\selfInd I\,\ddt{I}{t}. \end{equation} Integrating this equation, we find that the energy required from an external source to overcome the emf in the self-inductance while building up the current4 (which must equal the energy stored, $U$) is \begin{equation} \label{Eq:II:17:37} -W=U=\tfrac{1}{2}\selfInd I^2. \end{equation} Therefore the energy stored in an inductance is $\tfrac{1}{2}\selfInd I^2$.
Applying the same arguments to a pair of coils such as those in Figs. 17–8 or 17–9, we can show that the total electrical energy of the system is given by \begin{equation} \label{Eq:II:17:38} U=\tfrac{1}{2}\selfInd_1I_1^2+\tfrac{1}{2}\selfInd_2I_2^2+\mutualInd I_1I_2. \end{equation} For, starting with $I=0$ in both coils, we could first turn on the current $I_1$ in coil $1$, with $I_2=0$. The work done is just $\tfrac{1}{2}\selfInd_1I_1^2$. But now, on turning up $I_2$, we not only do the work $\tfrac{1}{2}\selfInd_2I_2^2$ against the emf in circuit $2$, but also an additional amount $\mutualInd I_1I_2$, which is the integral of the emf [$\mutualInd(dI_2/dt)$] in circuit $1$ times the now constant current $I_1$ in that circuit.
Suppose we now wish to find the force between any two coils carrying the currents $I_1$ and $I_2$. We might at first expect that we could use the principle of virtual work, by taking the change in the energy of Eq. (17.38). We must remember, of course, that as we change the relative positions of the coils the only quantity which varies is the mutual inductance $\mutualInd$. We might then write the equation of virtual work as \begin{equation*} -F\,\Delta x=\Delta U=I_1I_2\,\Delta\mutualInd\quad(\text{wrong}). \end{equation*} But this equation is wrong because, as we have seen earlier, it includes only the change in the energy of the two coils and not the change in the energy of the sources which are maintaining the currents $I_1$ and $I_2$ at their constant values. We can now understand that these sources must supply energy against the induced emf’s in the coils as they are moved. If we wish to apply the principle of virtual work correctly, we must also include these energies. As we have seen, however, we may take a short cut and use the principle of virtual work by remembering that the total energy is the negative of what we have called $U_{\text{mech}}$, the “mechanical energy.” We can therefore write for the force \begin{equation} \label{Eq:II:17:39} -F\,\Delta x=\Delta U_{\text{mech}}=-\Delta U. \end{equation} The force between two coils is then given by \begin{equation*} F\,\Delta x=I_1I_2\,\Delta\mutualInd. \end{equation*}
Equation (17.38) for the energy of a system of two coils can be used to show that an interesting inequality exists between mutual inductance $\mutualInd$ and the self-inductances $\selfInd_1$ and $\selfInd_2$ of the two coils. It is clear that the energy of two coils must be positive. If we begin with zero currents in the coils and increase these currents to some values, we have been adding energy to the system. If not, the currents would spontaneously increase with release of energy to the rest of the world—an unlikely thing to happen! Now our energy equation, Eq. (17.38), can equally well be written in the following form: \begin{equation} \label{Eq:II:17:40} U=\frac{1}{2}\,\selfInd_1\biggl(I_1+\frac{\mutualInd}{\selfInd_1}\,I_2\biggr)^2+ \frac{1}{2}\biggl(\selfInd_2-\frac{\mutualInd^2}{\selfInd_1}\biggr)I_2^2. \end{equation} That is just an algebraic transformation. This quantity must always be positive for any values of $I_1$ and $I_2$. In particular, it must be positive if $I_2$ should happen to have the special value \begin{equation} \label{Eq:II:17:41} I_2=-\frac{\selfInd_1}{\mutualInd}\,I_1. \end{equation} But with this current for $I_2$, the first term in Eq. (17.40) is zero. If the energy is to be positive, the last term in (17.40) must be greater than zero. We have the requirement that \begin{equation*} \selfInd_1\selfInd_2>\mutualInd^2. \end{equation*} We have thus proved the general result that the magnitude of the mutual inductance $\mutualInd$ of any two coils is necessarily less than or equal to the geometric mean of the two self-inductances. ($\mutualInd$ itself may be positive or negative, depending on the sign conventions for the currents $I_1$ and $I_2$.) \begin{equation} \label{Eq:II:17:42} \abs{\mutualInd}<\sqrt{\selfInd_1\selfInd_2}. \end{equation}
The relation between $\mutualInd$ and the self-inductances is usually written as \begin{equation} \label{Eq:II:17:43} \mutualInd=k\sqrt{\selfInd_1\selfInd_2}. \end{equation} The constant $k$ is called the coefficient of coupling. If most of the flux from one coil links the other coil, the coefficient of coupling is near one; we say the coils are “tightly coupled.” If the coils are far apart or otherwise arranged so that there is very little mutual flux linkage, the coefficient of coupling is near zero and the mutual inductance is very small.
For calculating the mutual inductance of two coils, we have given in Eq. (17.30) a formula which is a double line integral around the two circuits. We might think that the same formula could be used to get the self-inductance of a single coil by carrying out both line integrals around the same coil. This, however, will not work, because the denominator $r_{12}$ of the integrand will go to zero when the two line elements $d\FLPs_1$ and $d\FLPs_2$ are at the same point on the coil. The self-inductance obtained from this formula is infinite. The reason is that this formula is an approximation that is valid only when the cross sections of the wires of the two circuits are small compared with the distance from one circuit to the other. Clearly, this approximation doesn’t hold for a single coil. It is, in fact, true that the inductance of a single coil tends logarithmically to infinity as the diameter of its wire is made smaller and smaller.
We must, then, look for a different way of calculating the self-inductance of a single coil. It is necessary to take into account the distribution of the currents within the wires because the size of the wire is an important parameter. We should therefore ask not what is the inductance of a “circuit,” but what is the inductance of a distribution of conductors. Perhaps the easiest way to find this inductance is to make use of the magnetic energy. We found earlier, in Section 15–3, an expression for the magnetic energy of a distribution of stationary currents: \begin{equation} \label{Eq:II:17:44} U=\tfrac{1}{2}\int\FLPj\cdot\FLPA\,dV. \end{equation} If we know the distribution of current density $\FLPj$, we can compute the vector potential $\FLPA$ and then evaluate the integral of Eq. (17.44) to get the energy. This energy is equal to the magnetic energy of the self-inductance, $\tfrac{1}{2}\selfInd I^2$. Equating the two gives us a formula for the inductance: \begin{equation} \label{Eq:II:17:45} \selfInd=\frac{1}{I^2}\int\FLPj\cdot\FLPA\,dV. \end{equation} We expect, of course, that the inductance is a number depending only on the geometry of the circuit and not on the current $I$ in the circuit. The formula of Eq. (17.45) will indeed give such a result, because the integral in this equation is proportional to the square of the current—the current appears once through $\FLPj$ and again through the vector potential $\FLPA$. The integral divided by $I^2$ will depend on the geometry of the circuit but not on the current $I$.
Equation (17.44) for the energy of a current distribution can be put in a quite different form which is sometimes more convenient for calculation. Also, as we will see later, it is a form that is important because it is more generally valid. In the energy equation, Eq. (17.44), both $\FLPA$ and $\FLPj$ can be related to $\FLPB$, so we can hope to express the energy in terms of the magnetic field—just as we were able to relate the electrostatic energy to the electric field. We begin by replacing $\FLPj$ by $\epsO c^2\FLPcurl{\FLPB}$. We cannot replace $\FLPA$ so easily, since $\FLPB=\FLPcurl{\FLPA}$ cannot be reversed to give $\FLPA$ in terms of $\FLPB$. Anyway, we can write \begin{equation} \label{Eq:II:17:46} U=\frac{\epsO c^2}{2}\int(\FLPcurl{\FLPB})\cdot\FLPA\,dV. \end{equation}
The interesting thing is that—with some restrictions—this integral can be written as \begin{equation} \label{Eq:II:17:47} U=\frac{\epsO c^2}{2}\int\FLPB\cdot(\FLPcurl{\FLPA})\,dV. \end{equation} To see this, we write out in detail a typical term. Suppose that we take the term $(\FLPcurl{\FLPB})_zA_z$ which occurs in the integral of Eq. (17.46). Writing out the components, we get \begin{equation*} \int\biggl(\ddp{B_y}{x}-\ddp{B_x}{y}\biggr)A_z\,dx\,dy\,dz. \end{equation*} (There are, of course, two more integrals of the same kind.) We now integrate the first term with respect to $x$—integrating by parts. That is, we can say \begin{equation*} \int\ddp{B_y}{x}\,A_z\,dx=B_yA_z-\int B_y\,\ddp{A_z}{x}\,dx. \end{equation*} Now suppose that our system—meaning the sources and fields—is finite, so that as we go to large distances all fields go to zero. Then if the integrals are carried out over all space, evaluating the term $B_yA_z$ at the limits will give zero. We have left only the term with $B_y(\ddpl{A_z}{x})$, which is evidently one part of $B_y(\FLPcurl{\FLPA})_y$ and, therefore, of $\FLPB\cdot(\FLPcurl{\FLPA})$. If you work out the other five terms, you will see that Eq. (17.47) is indeed equivalent to Eq. (17.46).
But now we can replace $(\FLPcurl{\FLPA})$ by $\FLPB$, to get \begin{equation} \label{Eq:II:17:48} U=\frac{\epsO c^2}{2}\int\FLPB\cdot\FLPB\,dV. \end{equation} We have expressed the energy of a magnetostatic situation in terms of the magnetic field only. The expression corresponds closely to the formula we found for the electrostatic energy: \begin{equation} \label{Eq:II:17:49} U=\frac{\epsO}{2}\int\FLPE\cdot\FLPE\,dV. \end{equation}
One reason for emphasizing these two energy formulas is that sometimes they are more convenient to use. More important, it turns out that for dynamic fields (when $\FLPE$ and $\FLPB$ are changing with time) the two expressions (17.48) and (17.49) remain true, whereas the other formulas we have given for electric or magnetic energies are no longer correct—they hold only for static fields.
If we know the magnetic field $\FLPB$ of a single coil, we can find the self-inductance by equating the energy expression (17.48) to $\tfrac{1}{2}\selfInd I^2$. Let’s see how this works by finding the self-inductance of a long solenoid. We have seen earlier that the magnetic field inside a solenoid is uniform and $\FLPB$ outside is zero. The magnitude of the field inside is $B=nI/\epsO c^2$, where $n$ is the number of turns per unit length in the winding and $I$ is the current. If the radius of the coil is $r$ and its length is $L$ (we take $L$ very long, so that we can neglect end effects, i.e., $L\gg r$), the volume inside is $\pi r^2L$. The magnetic energy is therefore \begin{equation*} U=\frac{\epsO c^2}{2}\,B^2\cdot(\text{Vol})=\frac{n^2I^2}{2\epsO c^2}\, \pi r^2L, \end{equation*} which is equal to $\tfrac{1}{2}\selfInd I^2$. Or, \begin{equation} \label{Eq:II:17:50} \selfInd=\frac{\pi r^2n^2}{\epsO c^2}\,L. \end{equation}
- Now that we are using the letter $A$ for the vector potential, we prefer to let $S$ stand for a surface area. ↩
- The sign of $\mutualInd_{12}$ and $\mutualInd_{21}$ in Eqs. (17.31) and (17.32) depends on the arbitrary choices for the sense of a positive current in the two coils. ↩
- This is, incidentally, not the only way a correspondence can be set up between mechanical and electrical quantities. ↩
- We are neglecting any energy loss to heat from the current in the resistance of the coil. Such losses require additional energy from the source but do not change the energy which goes into the inductance. ↩