18
The Maxwell Equations
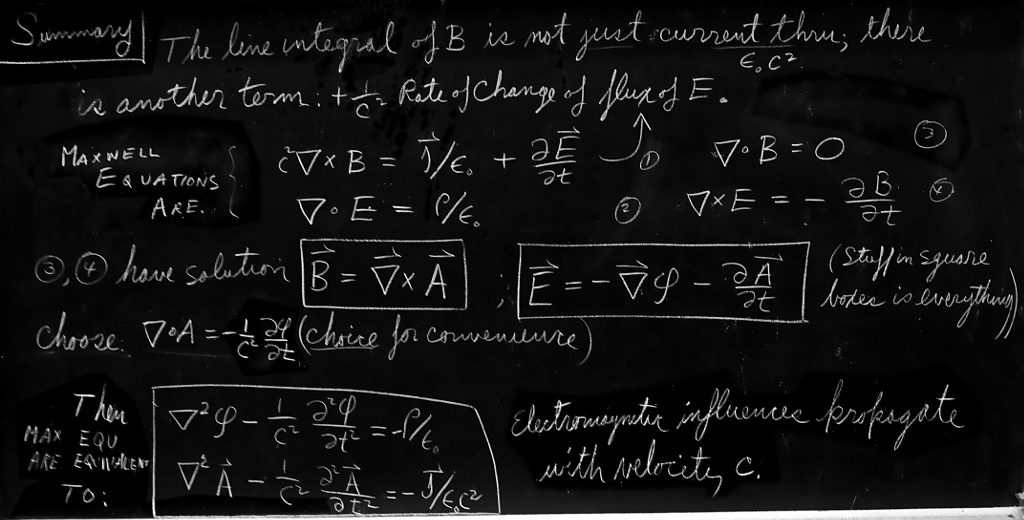
18–1Maxwell’s equations
In this chapter we come back to the complete set of the four Maxwell equations that we took as our starting point in Chapter 1. Until now, we have been studying Maxwell’s equations in bits and pieces; it is time to add one final piece, and to put them all together. We will then have the complete and correct story for electromagnetic fields that may be changing with time in any way. Anything said in this chapter that contradicts something said earlier is true and what was said earlier is false—because what was said earlier applied to such special situations as, for instance, steady currents or fixed charges. Although we have been very careful to point out the restrictions whenever we wrote an equation, it is easy to forget all of the qualifications and to learn too well the wrong equations. Now we are ready to give the whole truth, with no qualifications (or almost none).
The complete Maxwell equations are written in Table 18–1, in words as well as in mathematical symbols. The fact that the words are equivalent to the equations should by this time be familiar—you should be able to translate back and forth from one form to the other.
The first equation—that the divergence of $\FLPE$ is the charge density over $\epsO$—is true in general. In dynamic as well as in static fields, Gauss’ law is always valid. The flux of $\FLPE$ through any closed surface is proportional to the charge inside. The third equation is the corresponding general law for magnetic fields. Since there are no magnetic charges, the flux of $\FLPB$ through any closed surface is always zero. The second equation, that the curl of $\FLPE$ is $-\ddpl{\FLPB}{t}$, is Faraday’s law and was discussed in the last two chapters. It also is generally true. The last equation has something new. We have seen before only the part of it which holds for steady currents. In that case we said that the curl of $\FLPB$ is $\FLPj/\epsO c^2$, but the correct general equation has a new part that was discovered by Maxwell.
Until Maxwell’s work, the known laws of electricity and magnetism were those we have studied in Chapters 3 through 17. In particular, the equation for the magnetic field of steady currents was known only as \begin{equation} \label{Eq:II:18:1} \FLPcurl{\FLPB}=\frac{\FLPj}{\epsO c^2}. \end{equation} Maxwell began by considering these known laws and expressing them as differential equations, as we have done here. (Although the $\FLPnabla$ notation was not yet invented, it is mainly due to Maxwell that the importance of the combinations of derivatives, which we today call the curl and the divergence, first became apparent.) He then noticed that there was something strange about Eq. (18.1). If one takes the divergence of this equation, the left-hand side will be zero, because the divergence of a curl is always zero. So this equation requires that the divergence of $\FLPj$ also be zero. But if the divergence of $\FLPj$ is zero, then the total flux of current out of any closed surface is also zero.
The flux of current from a closed surface is the decrease of the charge inside the surface. This certainly cannot in general be zero because we know that the charges can be moved from one place to another. The equation \begin{equation} \label{Eq:II:18:2} \FLPdiv{\FLPj}=-\ddp{\rho}{t} \end{equation} has, in fact, been almost our definition of $\FLPj$. This equation expresses the very fundamental law that electric charge is conserved—any flow of charge must come from some supply. Maxwell appreciated this difficulty and proposed that it could be avoided by adding the term $\ddpl{\FLPE}{t}$ to the right-hand side of Eq. (18.1); he then got the fourth equation in Table 18–1: \begin{equation*} \text{IV.}\quad c^2\FLPcurl{\FLPB}=\frac{\FLPj}{\epsO}+\ddp{\FLPE}{t}. \end{equation*}
It was not yet customary in Maxwell’s time to think in terms of abstract fields. Maxwell discussed his ideas in terms of a model in which the vacuum was like an elastic solid. He also tried to explain the meaning of his new equation in terms of the mechanical model. There was much reluctance to accept his theory, first because of the model, and second because there was at first no experimental justification. Today, we understand better that what counts are the equations themselves and not the model used to get them. We may only question whether the equations are true or false. This is answered by doing experiments, and untold numbers of experiments have confirmed Maxwell’s equations. If we take away the scaffolding he used to build it, we find that Maxwell’s beautiful edifice stands on its own. He brought together all of the laws of electricity and magnetism and made one complete and beautiful theory.
2025.6.17: 파인만의 설명이 간략 명쾌하지만, A treatise on electricity & magnetism 1,2권을 들여다 보면
1. 수많은 사람들의 연구 노력들과
2. 그 결과들의 수학적 description 대응성에 대한 끊임없는 일관성 체크가 있었기 때문에
맥스웰로 하여금 위 4 방정식으로 압축하고 빛이 전자기파라는 걸 확신할 수 있도록 만든 거 => 리만의 대응성
3. 6.19: 더 나아가 소위 학자들의 경험/결과에만 국한시키지 말고
지난 수천년간 많은 사람들이 다양하게 감지한 것들을 일관성 있게 정리하면 더 넓고 깊게 자연을 들여다 볼 수 있다
2019-20:
위 방정식들을 맥스웰 방정식이라고 하는데, 각각 별도의 기여자 이름이 붙어있다. 도대체 맥스웰이(패러디의 제자) 한 게 뭔가라는 의문이 들었었는데...
맥스웰은
(1) 패러디 발견을 18.1식과 같은 수학적 표현으로 정리하고 암페어 법칙도 On Physical Lines of Force에서(1861년)
도출해내고(출처: 암페어 법칙과 맥스웰)
간단한 I, II와 달리, 복잡한 III, IV를 수학적 표현해 냈다는 자체가 대단한 업적.
그로부터 수학적으로
(2) 위 파인만 설명한 대로 모순 발견. 전기, 자기의 missing link 발견한 것(비교: 마이켈슨-몰리 실험결과를 설명하기 위한 로렌쯔 전환 고안 그리고 Galois가 5차 이상 방정식의 radical solution 관련 field를 sovable group에 대응시킨 것 등)
(3) 그리고 전자기 포텐셜이 빛의 속도 파동방정식 만족하니 빛이라고 단정한 거. 파동에 매질(ether) 존재에 집착하다 아인슈타인 이후로 내버림.
* 위 4개 방정식으로 맥스웰이 정리하기까지 가우스, 리만, 포아송(Poisson), 그린(G. Green) 등 많은 사람들이 연구하였다. 그 중에서 포아송 연구를 더 발전시킨 그린이 1828년 'An Essay on the Application of Mathematical Analysis to the Theories of Electricity and Magnetism'에 발표한 수학적 결과가 그린 정리(Green' theorem)
맥스웰이 'A treatise on electricity & magnetism' 1권(p. 126)에서 정리 증명(Green function과 함께)을 다루었지만, 역시나 쉽고 직관적인 증명은 파인만의 파인만 강의 II, 3-9에 있다, 그린 정리의 일반화/확장인 Stoke's theorem까지 덤으로
=> 그린 정리 증명
Let us show that the extra term is just what is required to straighten out the difficulty Maxwell discovered. Taking the divergence of his equation (IV in Table 18–1), we must have that the divergence of the right-hand side is zero: \begin{equation} \label{Eq:II:18:3} \FLPdiv{\frac{\FLPj}{\epsO}}+\FLPdiv{\ddp{\FLPE}{t}}=0. \end{equation} In the second term, the order of the derivatives with respect to coordinates and time can be reversed, so the equation can be rewritten as \begin{equation} \label{Eq:II:18:4} \FLPdiv{\FLPj}+\epsO\,\ddp{}{t}\,\FLPdiv{\FLPE}=0. \end{equation} But the first of Maxwell’s equations says that the divergence of $\FLPE$ is $\rho/\epsO$. Inserting this equality in Eq. (18.4), we get back Eq. (18.2), which we know is true. Conversely, if we accept Maxwell’s equations—and we do because no one has ever found an experiment that disagrees with them—we must conclude that charge is always conserved.
The laws of physics have no answer to the question: “What happens if a charge is suddenly created at this point—what electromagnetic effects are produced?” No answer can be given because our equations say it doesn’t happen. If it were to happen, we would need new laws, but we cannot say what they would be. We have not had the chance to observe how a world without charge conservation behaves. According to our equations, if you suddenly place a charge at some point, you had to carry it there from somewhere else. In that case, we can say what would happen.
When we added a new term to the equation for the curl of $\FLPE$, we found that a whole new class of phenomena was described. We shall see that Maxwell’s little addition to the equation for $\FLPcurl{\FLPB}$ also has far-reaching consequences. We can touch on only a few of them in this chapter.
18–2How the new term works
As our first example we consider what happens with a spherically symmetric radial distribution of current. Suppose we imagine a little sphere with radioactive material on it. This radioactive material is squirting out some charged particles. (Or we could imagine a large block of jello with a small hole in the center into which some charge had been injected with a hypodermic needle and from which the charge is slowly leaking out.) In either case we would have a current that is everywhere radially outward. We will assume that it has the same magnitude in all directions.
Let the total charge inside any radius $r$ be $Q(r)$. If the radial current density at the same radius is $\FLPj(r)$, then Eq. (18.2) requires that $Q$ decreases at the rate \begin{equation} \label{Eq:II:18:5} \ddp{Q(r)}{t}=-4\pi r^2j(r). \end{equation}
We now ask about the magnetic field produced by the currents in this situation. Suppose we draw some loop $\Gamma$ on a sphere of radius $r$, as shown in Fig. 18–1. There is some current through this loop, so we might expect to find a magnetic field circulating in the direction shown.
But we are already in difficulty. How can the $\FLPB$ have any particular direction on the sphere? A different choice of $\Gamma$ would allow us to conclude that its direction is exactly opposite to that shown. So how can there be any circulation of $\FLPB$ around the currents?
We are saved by Maxwell’s equation. The circulation of $\FLPB$ depends not only on the total current through $\Gamma$ but also on the rate of change with time of the electric flux through it. It must be that these two parts just cancel. Let’s see if that works out.
The electric field at the radius $r$ must be $Q(r)/4\pi\epsO r^2$—so long as the charge is symmetrically distributed, as we assume. It is radial, and its rate of change is then \begin{equation} \label{Eq:II:18:6} \ddp{E}{t}=\frac{1}{4\pi\epsO r^2}\,\ddp{Q}{t}. \end{equation} Comparing this with Eq. (18.5), we see \begin{equation} \label{Eq:II:18:7} \ddp{E}{t}=-\frac{j}{\epsO}. \end{equation} In Eq. IV the two source terms cancel and the curl of $\FLPB$ is always zero. There is no magnetic field in our example.
As our second example, we consider the magnetic field of a wire used to charge a parallel-plate condenser (see Fig. 18–2). If the charge $Q$ on the plates is changing with time (but not too fast), the current in the wires is equal to $dQ/dt$. We would expect that this current will produce a magnetic field that encircles the wire. Surely, the current close to the plate must produce the normal magnetic field—it cannot depend on where the current is going.
Suppose we take a loop $\Gamma_1$ which is a circle with radius $r$, as shown in part (a) of the figure. The line integral of the magnetic field should be equal to the current $I$ divided by $\epsO c^2$. We have \begin{equation} \label{Eq:II:18:8} 2\pi rB=\frac{I}{\epsO c^2}. \end{equation} This is what we would get for a steady current, but it is also correct with Maxwell’s addition, because if we consider the plane surface $S$ inside the circle, there are no electric fields on it (assuming the wire to be a very good conductor). The surface integral of $\ddpl{\FLPE}{t}$ is zero.
Suppose, however, that we now slowly move the curve $\Gamma$ downward. We get always the same result until we draw even with the plates of the condenser. Then the current $I$ goes to zero. Does the magnetic field disappear? That would be quite strange. Let’s see what Maxwell’s equation says for the curve $\Gamma_2$, which is a circle of radius $r$ whose plane passes between the condenser plates [Fig. 18–2(b)]. The line integral of $\FLPB$ around $\Gamma_2$ is $2\pi rB$. This must equal the time derivative of the flux of $\FLPE$ through the plane circular surface $S_2$. This flux of $\FLPE$, we know from Gauss’ law, must be equal to $1/\epsO$ times the charge $Q$ on one of the condenser plates. We have \begin{equation} \label{Eq:II:18:9} c^2\,2\pi rB=\ddt{}{t}\biggl(\frac{Q}{\epsO}\biggr). \end{equation}
That is very convenient. It is the same result we found in Eq. (18.8). Integrating over the changing electric field gives the same magnetic field as does integrating over the current in the wire.
2025.3.23: 기, 에너지 보존 법칙
Of course, that is just what Maxwell’s equation says. It is easy to see that this must always be so by applying our same arguments to the two surfaces $S_1$ and $S_1'$ that are bounded by the same circle $\Gamma_1$ in Fig. 18–2(b). Through $S_1$ there is the current $I$, but no electric flux. Through $S_1'$ there is no current, but an electric flux changing at the rate $I/\epsO$. The same $\FLPB$ is obtained if we use Eq. IV with either surface.From our discussion so far of Maxwell’s new term, you may have the impression that it doesn’t add much—that it just fixes up the equations to agree with what we already expect. It is true that if we just consider Eq. IV by itself, nothing particularly new comes out. The words “by itself” are, however, all-important. Maxwell’s small change in Eq. IV, when combined with the other equations, does indeed produce much that is new and important. Before we take up these matters, however, we want to speak more about Table 18–1.
18–3All of classical physics
In Table 18–1 we have all that was known of fundamental classical physics, that is, the physics that was known by 1905. Here it all is, in one table. With these equations we can understand the complete realm of classical physics.
First we have the Maxwell equations—written in both the expanded form and the short mathematical form. Then there is the conservation of charge, which is even written in parentheses, because the moment we have the complete Maxwell equations, we can deduce from them the conservation of charge. So the table is even a little redundant. Next, we have written the force law, because having all the electric and magnetic fields doesn’t tell us anything until we know what they do to charges. Knowing $\FLPE$ and $\FLPB$, however, we can find the force on an object with the charge $q$ moving with velocity $\FLPv$. Finally, having the force doesn’t tell us anything until we know what happens when a force pushes on something; we need the law of motion, which is that the force is equal to the rate of change of the momentum. (Remember? We had that in Volume I.) We even include relativity effects by writing the momentum as $\FLPp=m_0\FLPv/\sqrt{1-v^2/c^2}$.
If we really want to be complete, we should add one more law—Newton’s law of gravitation—so we put that at the end.
Therefore in one small table we have all the fundamental laws of classical physics—even with room to write them out in words and with some redundancy. This is a great moment. We have climbed a great peak. We are on the top of K2—we are nearly ready for Mount Everest, which is quantum mechanics. We have climbed the peak of a “Great Divide,” and now we can go down the other side.
We have mainly been trying to learn how to understand the equations. Now that we have the whole thing put together, we are going to study what the equations mean—what new things they say that we haven’t already seen. We’ve been working hard to get up to this point. It has been a great effort, but now we are going to have nice coasting downhill as we see all the consequences of our accomplishment.
18–4A travelling field
Now for the new consequences. They come from putting together all of Maxwell’s equations. First, let’s see what would happen in a circumstance which we pick to be particularly simple. By assuming that all the quantities vary only in one coordinate, we will have a one-dimensional problem. The situation is shown in Fig. 18–3. We have a sheet of charge located on the $yz$-plane. The sheet is first at rest, then instantaneously given a velocity $u$ in the $y$-direction, and kept moving with this constant velocity. You might worry about having such an “infinite” acceleration, but it doesn’t really matter; just imagine that the velocity is brought to $u$ very quickly. So we have suddenly a surface current $J$ ($J$ is the current per unit width in the $z$-direction). To keep the problem simple, we suppose that there is also a stationary sheet of charge of opposite sign superposed on the $yz$-plane, so that there are no electrostatic effects. Also, although in the figure we show only what is happening in a finite region, we imagine that the sheet extends to infinity in $\pm y$ and $\pm z$. In other words, we have a situation where there is no current, and then suddenly there is a uniform sheet of current. What will happen?
Well, when there is a sheet of current in the plus $y$-direction, there is, as we know, a magnetic field generated which will be in the minus $z$-direction for $x>0$ and in the opposite direction for $x<0$. We could find the magnitude of $\FLPB$ by using the fact that the line integral of the magnetic field will be equal to the current over $\epsO c^2$. We would get that $B=J/2\epsO c^2$ (since the current $I$ in a strip of width $w$ is $Jw$ and the line integral of $\FLPB$ is $2Bw$).
This gives us the field next to the sheet—for small $x$—but since we are imagining an infinite sheet, we would expect the same argument to give the magnetic field farther out for larger values of $x$. However, that would mean that the moment we turn on the current, the magnetic field is suddenly changed from zero to a finite value everywhere. But wait! If the magnetic field is suddenly changed, it will produce tremendous electrical effects. (If it changes in any way, there are electrical effects.) So because we moved the sheet of charge, we make a changing magnetic field, and therefore electric fields must be generated. If there are electric fields generated, they had to start from zero and change to something else. There will be some $\ddpl{\FLPE}{t}$ that will make a contribution, together with the current $J$, to the production of the magnetic field. So through the various equations there is a big intermixing, and we have to try to solve for all the fields at once.
By looking at the Maxwell equations alone, it is not easy to see directly how to get the solution. So we will first show you what the answer is and then verify that it does indeed satisfy the equations. The answer is the following: The field $\FLPB$ that we computed is, in fact, generated right next to the current sheet (for small $x$). It must be so, because if we make a tiny loop around the sheet, there is no room for any electric flux to go through it. But the field $\FLPB$ out farther—for larger $x$—is, at first, zero. It stays zero for awhile, and then suddenly turns on. In short, we turn on the current and the magnetic field immediately next to it turns on to a constant value $\FLPB$; then the turning on of $\FLPB$ spreads out from the source region. After a certain time, there is a uniform magnetic field everywhere out to some value $x$, and then zero beyond. Because of the symmetry, it spreads in both the plus and minus $x$-directions.
2022.10.16: 현상들을 제대로 이해하기 위해서는, 수학적 형식 같은 거에 얽매이지 말고 결과와 부합하는 방향으로 이해하는 노력이 중요하다.
따로따로 보면 서로 상충되는 모순이 보이는 현 세계상황도, 궁극적 목적 달성하기 위한 사이드쇼로 이해되는 것처럼.
10.17: 답이 대강 어떻다는 걸 알고 진동 방정식 풀듯이 풀어가는 중.
2024.7.15:
1. 전자기장 wavefront에 대한 상황 통찰로
2. 전류가 흐르면 instantly 자기장 생기고(그 사이에 no room for 전기장) 곧 이어 전기장 발생한다는 사실과
3. 맥스웰 방정식과 뉴튼식 접근 방법을(uniform field) 절묘하게 배합하여 그럴듯한 설명하는 것이 연구 방법이란 걸 파인만이 보여준다
The $\FLPE$-field does the same thing. Before $t=0$ (when we turn on the current), the field is zero everywhere. Then after the time $t$, both $\FLPE$ and $\FLPB$ are uniform out to the distance $x=vt$, and zero beyond.
* 2021.8.25: 자기장 변화에 의해 전기장 발생. unit time 동안의 그 전기장은 일정, 즉 uniform, 이게 뉴튼의 물리 사고. 미적분은 그 산물.
The fields make their way forward like a tidal wave, with a front moving at a uniform velocity which turns out to be $c$, but for a while we will just call it $v$. A graph of the magnitude of $\FLPE$ or $\FLPB$ versus $x$, as they appear at the time $t$, is shown in Fig. 18–4(a). Looking again at Fig. 18–3, at the time $t$, the region between $x=\pm vt$ is “filled” with the fields, but they have not yet reached beyond. We emphasize again that we are assuming that the current sheet and, therefore the fields $\FLPE$ and $\FLPB$, extend infinitely far in both the $y$- and $z$-directions. (We cannot draw an infinite sheet, so we have shown only what happens in a finite area.)We want now to analyze quantitatively what is happening. To do that, we want to look at two cross-sectional views, a top view looking down along the $y$-axis, as shown in Fig. 18–5, and a side view looking back along the $z$-axis, as shown in Fig. 18–6. Suppose we start with the side view. We see the charged sheet moving up; the magnetic field points into the page for $+x$, and out of the page for $-x$, and the electric field is downward everywhere—out to $x=\pm vt$.
Let’s see if these fields are consistent with Maxwell’s equations. Let’s first draw one of those loops that we use to calculate a line integral, say the rectangle $\Gamma_2$ shown in Fig. 18–6. You notice that one side of the rectangle is in the region where there are fields, but one side is in the region the fields have still not reached. There is some magnetic flux through this loop. If it is changing, there should be an emf around it. If the wavefront is moving, we will have a changing magnetic flux, because the area in which $\FLPB$ exists is progressively increasing at the velocity $v$. The flux inside $\Gamma_2$ is $B$ times the part of the area inside $\Gamma_2$ which has a magnetic field. The rate of change of the flux, since the magnitude of $\FLPB$ is constant, is the magnitude times the rate of change of the area. The rate of change of the area is easy. If the width of the rectangle $\Gamma_2$ is $L$, the area in which $\FLPB$ exists changes by $Lv\,\Delta t$ in the time $\Delta t$. (See Fig. 18–6.) The rate of change of flux is then $BLv$. According to Faraday’s law, this should equal minus the line integral of $\FLPE$ around $\Gamma_2$, which is just $EL$. We have the equation \begin{equation} \label{Eq:II:18:10} E=vB. \end{equation} So if the ratio of $E$ to $B$ is $v$, the fields we have assumed will satisfy Faraday’s equation.
But that is not the only equation; we have the other equation relating $\FLPE$ and $\FLPB$: \begin{equation} \label{Eq:II:18:11} c^2\FLPcurl{\FLPB}=\frac{\FLPj}{\epsO}+\ddp{\FLPE}{t}. \end{equation} To apply this equation, we look at the top view in Fig. 18–5. We have seen that this equation will give us the value of $B$ next to the current sheet. Also, for any loop drawn outside the sheet but behind the wavefront, there is no curl of $\FLPB$ nor any $\FLPj$ or changing $\FLPE$, so the equation is correct there. Now let’s look at what happens for the curve $\Gamma_1$ that intersects the wavefront, as shown in Fig. 18–5. Here there are no currents, so Eq. (18.11) can be written—in integral form—as \begin{equation} \label{Eq:II:18:12} c^2\oint_{\Gamma_1}\FLPB\cdot d\FLPs=\ddt{}{t}\!\! \underset{\text{inside $\Gamma_1$}}{\int} \!\FLPE\cdot\FLPn\,da. \end{equation}
2025.3.18: This is wrong! 전자 흐름이 왜 sheet에만 존재해야 하는가? 결과에 꿰맞추기 쉬워서?
전자와 hole, 즉 기 장벽에 고차원으로의 관문이 열렸다 닫히는 것, 기의 부대낌에 의해 빵구나서.
전자기파가 나비처럼 홀로 퍼지는 건 홀과 전자를 바느질의 overlock 내지 용수철 바인더처럼 고차원으로 드나들며 전진하는 거.
3.23: 관문 전자 통과한 일부는 곧바로 전진하고 대다수는 circular 운동하면서 부대끼니 원주를 따라 관문이 생기고 그 관문을 통과한 대다수 기들이 형성한 원들
즉, 토러스 형태의 기들 모임이 자기장이라는 것
부차적으로 든 perpetual motion
1. 슈레딩거 방정식이 말해주듯이, 기 움직임은 주변기와의 세기 차이($-\frac{\hbar^2}{2m}\nabla^2\psi$)와 밀침($V\psi$) 효과에 의하고
2. 3차원에서는 Carnot의 가정처럼 불가능하지만, 전진한 '앞기'가 다른 차원을 통해 '뒷기'의 뒤에 위치한다면 외부기 도움 필요 없이 가능
3. 기 행태
We have a solution in which we have a constant $\FLPB$ and a constant $\FLPE$ behind the front, both at right angles to the direction in which the front is moving and at right angles to each other. Maxwell’s equations specify the ratio of $E$ to $B$. From Eqs. (18.10) and (18.13), \begin{equation*} E=vB,\quad\text{and}\quad E=\frac{c^2}{v}\,B. \end{equation*} But one moment! We have found two different conditions on the ratio $E/B$. Can such a field as we describe really exist? There is, of course, only one velocity $v$ for which both of these equations can hold, namely $v=c$. The wavefront must travel with the velocity $c$. We have an example in which the electrical influence from a current propagates at a certain finite velocity $c$.
Now let’s ask what happens if we suddenly stop the motion of the charged sheet after it has been on for a short time $T$. We can see what will happen by the principle of superposition. We had a current that was zero and then was suddenly turned on. We know the solution for that case. Now we are going to add another set of fields. We take another charged sheet and suddenly start it moving, in the opposite direction with the same speed, only at the time $T$ after we started the first current. The total current of the two added together is first zero, then on for a time $T$, then off again—because the two currents cancel. We have a square “pulse” of current.
The new negative current produces the same fields as the positive one, only with all the signs reversed and, of course, delayed in time by $T$. A wavefront again travels out at the velocity $c$. At the time $t$ it has reached the distance $x=\pm c(t-T)$, as shown in Fig. 18–4(b). So we have two “blocks” of field marching out at the speed $c$, as in parts (a) and (b) of Fig. 18–4. The combined fields are as shown in part (c) of the figure. The fields are zero for $x>ct$, they are constant (with the values we found above) between $x=c(t-T)$ and $x=ct$, and again zero for $x<c(t - T)$.
In short, we have a little piece of field—a block of thickness $cT$—which has left the current sheet and is travelling through space all by itself. The fields have “taken off”; they are propagating freely through space, no longer connected in any way with the source. The caterpillar has turned into a butterfly!
How can this bundle of electric and magnetic fields maintain itself? The answer is: by the combined effects of the Faraday law, $\FLPcurl{\FLPE}=-\ddpl{\FLPB}{t}$, and the new term of Maxwell, $c^2\FLPcurl{\FLPB}=\ddpl{\FLPE}{t}$. They cannot help maintaining themselves. Suppose the magnetic field were to disappear. There would be a changing magnetic field which would produce an electric field. If this electric field tries to go away, the changing electric field would create a magnetic field back again. So by a perpetual interplay—by the swishing back and forth from one field to the other—they must go on forever. It is impossible for them to disappear.1 They maintain themselves in a kind of a dance—one making the other, the second making the first—propagating onward through space.
18–5The speed of light
We have a wave which leaves the material source and goes outward at the velocity $c$, which is the speed of light. But let’s go back a moment. From a historical point of view, it wasn’t known that the coefficient $c$ in Maxwell’s equations was also the speed of light propagation. There was just a constant in the equations. We have called it $c$ from the beginning, because we knew what it would turn out to be. We didn’t think it would be sensible to make you learn the formulas with a different constant and then go back to substitute $c$ wherever it belonged. From the point of view of electricity and magnetism, however, we just start out with two constants, $\epsO$ and $c^2$, that appear in the equations of electrostatics and magnetostatics: \begin{equation} \label{Eq:II:18:14} \FLPdiv{\FLPE} =\frac{\rho}{\epsO} \end{equation} and \begin{equation} \label{Eq:II:18:15} \FLPcurl{\FLPB} =\frac{\FLPj}{\epsO c^2}. \end{equation} If we take any arbitrary definition of a unit of charge, we can determine experimentally the constant $\epsO$ required in Eq. (18.14)—say by measuring the force between two unit charges at rest, using Coulomb’s law. We must also determine experimentally the constant $\epsO c^2$ that appears in Eq. (18.15), which we can do, say, by measuring the force between two unit currents. (A unit current means one unit of charge per second.) The ratio of these two experimental constants is $c^2$—just another “electromagnetic constant.”
Notice now that this constant $c^2$ is the same no matter what we choose for our unit of charge. If we put twice as much “charge”—say twice as many proton charges—in our “unit” of charge, $\epsO$ would need to be one-fourth as large. When we pass two of these “unit” currents through two wires, there will be twice as much “charge” per second in each wire, so the force between two wires is four times larger. The constant $\epsO c^2$ must be reduced by one-fourth. But the ratio $\epsO c^2/\epsO$ is unchanged.
So just by experiments with charges and currents we find a number $c^2$ which turns out to be the square of the velocity of propagation of electromagnetic influences. From static measurements—by measuring the forces between two unit charges and between two unit currents—we find that $c=3.00\times10^8$ meters/sec. When Maxwell first made this calculation with his equations, he said that bundles of electric and magnetic fields should be propagated at this speed. He also remarked on the mysterious coincidence that this was the same as the speed of light. “We can scarcely avoid the inference,” said Maxwell, “that light consists in the transverse undulations of the same medium which is the cause of electric and magnetic phenomena.”
2024.7.14: 학문적 맘가짐: 수학은 자연 현상 이해하려는 물리에서 발전한 simulation
1. the simplest might be the right one
2. 따로 떨어져 보이는 흩어진 정보들로부터 연관성을 발견하여 전체적 그림 그리는 것, 예: 기의 흐름
3. 인간 아이디어와 물질 연관성 대응(7.18)
Maxwell had made one of the great unifications of physics. Before his time, there was light, and there was electricity and magnetism. The latter two had been unified by the experimental work of Faraday, Oersted, and Ampère. Then, all of a sudden, light was no longer “something else,” but was only electricity and magnetism in this new form—little pieces of electric and magnetic fields which propagate through space on their own.
We have called your attention to some characteristics of this special solution, which turn out to be true, however, for any electromagnetic wave: that the magnetic field is perpendicular to the direction of motion of the wavefront; that the electric field is likewise perpendicular to the direction of motion of the wavefront; and that the two vectors $\FLPE$ and $\FLPB$ are perpendicular to each other. Furthermore, the magnitude of the electric field $E$ is equal to $c$ times the magnitude of the magnetic field $B$. These three facts—that the two fields are transverse to the direction of propagation, that $\FLPB$ is perpendicular to $\FLPE$, and that $E=cB$—are generally true for any electromagnetic wave. Our special case is a good one—it shows all the main features of electromagnetic waves.
18–6Solving Maxwell’s equations; the potentials and the wave equation
Now we would like to do something mathematical; we want to write Maxwell’s equations in a simpler form. You may consider that we are complicating them, but if you will be patient a little bit, they will suddenly come out simpler. Although by this time you are thoroughly used to each of the Maxwell equations, there are many pieces that must all be put together. That’s what we want to do.
We begin with $\FLPdiv{\FLPB}=0$—the simplest of the equations. We know that it implies that $\FLPB$ is the curl of something. So, if we write \begin{equation} \label{Eq:II:18:16} \FLPB=\FLPcurl{\FLPA}, \end{equation} we have already solved one of Maxwell’s equations. (Incidentally, you appreciate that it remains true that another vector $\FLPA'$ would be just as good if $\FLPA'=\FLPA+\FLPgrad{\psi}$—where $\psi$ is any scalar field—because the curl of $\FLPgrad{\psi}$ is zero, and $\FLPB$ is still the same. We have talked about that before.)
We take next the Faraday law, $\FLPcurl{\FLPE}=-\ddpl{\FLPB}{t}$, because it doesn’t involve any currents or charges. If we write $\FLPB$ as $\FLPcurl{\FLPA}$ and differentiate with respect to $t$, we can write Faraday’s law in the form \begin{equation*} \FLPcurl{\FLPE}=-\ddp{}{t}\,\FLPcurl{\FLPA}. \end{equation*} Since we can differentiate either with respect to time or to space first, we can also write this equation as \begin{equation} \label{Eq:II:18:17} \FLPcurl{\biggl(\FLPE+\ddp{\FLPA}{t}\biggr)}=\FLPzero. \end{equation} We see that $\FLPE+\ddpl{\FLPA}{t}$ is a vector whose curl is equal to zero. Therefore that vector is the gradient of something. When we worked on electrostatics, we had $\FLPcurl{\FLPE}=\FLPzero$, and then we decided that $\FLPE$ itself was the gradient of something. We took it to be the gradient of $-\phi$ (the minus for technical convenience). We do the same thing for $\FLPE+\ddpl{\FLPA}{t}$; we set \begin{equation} \label{Eq:II:18:18} \FLPE+\ddp{\FLPA}{t}=-\FLPgrad{\phi}. \end{equation}
2025.4.3: $-\FLPgrad{\phi}$는 기존의 $E$와 steady하지 않은 위층의 기가 spiral down한 것의 합이라는 얘기
4.7: 위아래로 내려온 만큼 올라가고 올라간 만큼 내려온다는... 에너지/운동량 보존 원칙 그 맥락
We have solved two of Maxwell’s equations already, and we have found that to describe the electromagnetic fields $\FLPE$ and $\FLPB$, we need four potential functions: a scalar potential $\phi$ and a vector potential $\FLPA$, which is, of course, three functions.
Now that $\FLPA$ determines part of $\FLPE$, as well as $\FLPB$, what happens when we change $\FLPA$ to $\FLPA'=\FLPA+\FLPgrad{\psi}$? In general, $\FLPE$ would change if we didn’t take some special precaution. We can, however, still allow $\FLPA$ to be changed in this way without affecting the fields $\FLPE$ and $\FLPB$—that is, without changing the physics—if we always change $\FLPA$ and $\phi$ together by the rules \begin{equation} \label{Eq:II:18:20} \FLPA'=\FLPA+\FLPgrad{\psi},\quad \phi'=\phi-\ddp{\psi}{t}. \end{equation} Then neither $\FLPB$ nor $\FLPE$, obtained from Eq. (18.19), is changed.
Previously, we chose to make $\FLPdiv{\FLPA}=0$, to make the equations of statics somewhat simpler. We are not going to do that now; we are going to make a different choice. But we’ll wait a bit before saying what the choice is, because later it will be clear why the choice is made.
2024.6.25: 수학은 자연 현상 설명을 위한 도구, assumption or condition change
1.12: 2개의 전자기파 circular action은 $\phi, \psi$의 움직임
1.16: 고차원의 vector 포텐셜과 3차원 $B$의 missing link - 전자 model
(1) 수학적 link <= Stokes 정리
(2) 3차원에 미치는 실질적 영향
* 고차원으로부터 3차원에의 프로젝션이 curl product? ... halo
Now we return to the two remaining Maxwell equations which will give us relations between the potentials and the sources $\rho$ and $\FLPj$. Once we can determine $\FLPA$ and $\phi$ from the currents and charges, we can always get $\FLPE$ and $\FLPB$ from Eqs. (18.16) and (18.19), so we will have another form of Maxwell’s equations.
We begin by substituting Eq. (18.19) into $\FLPdiv{\FLPE}=\rho/\epsO$; we get \begin{equation*} \FLPdiv{\biggl(-\FLPgrad{\phi}-\ddp{\FLPA}{t}\biggr)}=\frac{\rho}{\epsO}, \end{equation*} which we can write also as \begin{equation} \label{Eq:II:18:21} -\nabla^2\phi-\ddp{}{t}\,\FLPdiv{\FLPA}=\frac{\rho}{\epsO}. \end{equation} This is one equation relating $\phi$ and $\FLPA$ to the sources.
Our final equation will be the most complicated. We start by rewriting the fourth Maxwell equation as \begin{equation*} c^2\FLPcurl{\FLPB}-\ddp{\FLPE}{t}=\frac{\FLPj}{\epsO}, \end{equation*} and then substitute for $\FLPB$ and $\FLPE$ in terms of the potentials, using Eqs. (18.16) and (18.19): \begin{equation*} c^2\FLPcurl{(\FLPcurl{\FLPA})}-\ddp{}{t}\, \biggl(-\FLPgrad{\phi}-\ddp{\FLPA}{t}\biggr)= \frac{\FLPj}{\epsO}. \end{equation*} The first term can be rewritten using the algebraic identity: $\FLPcurl{(\FLPcurl{\FLPA})}=$ $\FLPgrad{(\FLPdiv{\FLPA})}-\nabla^2\FLPA$; we get \begin{equation} \label{Eq:II:18:22} -c^2\nabla^2\FLPA+c^2\FLPgrad{(\FLPdiv{\FLPA})}+ \ddp{}{t}\,\FLPgrad{\phi}+\frac{\partial^2\FLPA}{\partial t^2}= \frac{\FLPj}{\epsO}. \end{equation} \begin{align} -c^2\nabla^2\FLPA&+c^2\FLPgrad{(\FLPdiv{\FLPA})}\notag\\[.5ex] \label{Eq:II:18:22} &+\ddp{}{t}\,\FLPgrad{\phi}+\frac{\partial^2\FLPA}{\partial t^2}= \frac{\FLPj}{\epsO}. \end{align} It’s not very simple!
Fortunately, we can now make use of our freedom to choose arbitrarily the divergence of $\FLPA$. What we are going to do is to use our choice to fix things so that the equations for $\FLPA$ and for $\phi$ are separated but have the same form. We can do this by taking2 \begin{equation} \label{Eq:II:18:23} \FLPdiv{\FLPA}=-\frac{1}{c^2}\,\ddp{\phi}{t}. \end{equation}
2025.4.17: 3차원의 포텐셜 변화가 고차원 기 변화와 같다는 거, 일종의 기 양 보존 방정식. relativity란 결국 기의 이동 변화를 말하는 거
When we do that, the two middle terms in $\FLPA$ and $\phi$ in Eq. (18.22) cancel, and that equation becomes much simpler: \begin{equation} \label{Eq:II:18:24} \nabla^2\FLPA-\frac{1}{c^2}\,\frac{\partial^2\FLPA}{\partial t^2}= -\frac{\FLPj}{\epsO c^2}. \end{equation} And our equation for $\phi$—Eq. (18.21)—takes on the same form: \begin{equation} \label{Eq:II:18:25} \nabla^2\phi-\frac{1}{c^2}\,\frac{\partial^2\phi}{\partial t^2}= -\frac{\rho}{\epsO}. \end{equation}What a beautiful set of equations! They are beautiful, first, because they are nicely separated—with the charge density, goes $\phi$; with the current, goes $\FLPA$.
2025.4.18: 2개 파동 방정식은 18.23으로 직결되어 있다. 그렇기에 방정식 II imply하듯 $\phi, E$가 증감할 때 $A, B$는 감증하는 거고
4.19: $\phi$의 변화가 없는 스테디한 경우는 정전기장만 있을뿐,
$E=E_{정전기장}+E_{\text{dynamic장}}$ <= 방정식 18.19, 잔잔한 수면의 dynamic 변화의 irregularity는 파도처럼 퍼진다
4.20: 아래 3층의 늘어난 기($\phi$)가 spiral up하니 위 3축의 기($A$)가 아래 3차원으로 스파이럴 다운한 거지, 굴러온 돌이 박힌 돌 내쳐지듯
1. $\phi$ 증감=$A$ 감소로 이어진다는 Faraday 발견(+ 참조)
2. 전자 구조틀의 toral action과
3. 기 보존식으로 부터 나오는 결론...
시간과 거리는 유니트만 다른 같은 거+ 방정식(18-24, 18-25)으로부터 기 보존이 확실 -4.21
4. 수학적 표현의 변화율에서 $\frac{1}{c^2}$ 차이는
가로지르는 전기장과 달리 전자장은 toroidal helix 만들며 빙돌아 나오기에
overlock, 뭉치듯 웅크리니 직접적으로 주변 기에 미치는 영향도 양에 비해 약한 거- 4.23&24:...
2025.4.21: diffrent unit but same thing, time= rate of structure forming => 기 보존식
Maxwell’s equations have led us to a new kind of equation for the potentials $\phi$ and $\FLPA$ but to the same mathematical form for all four functions $\phi$, $A_x$, $A_y$, and $A_z$. Once we learn how to solve these equations, we can get $\FLPB$ and $\FLPE$ from $\FLPcurl{\FLPA}$ and $-\FLPgrad{\phi}-\ddpl{\FLPA}{t}$. We have another form of the electromagnetic laws exactly equivalent to Maxwell’s equations, and in many situations they are much simpler to handle.
We have, in fact, already solved an equation much like Eq. (18.26). When we studied sound in Chapter 47 of Vol. I, we had an equation of the form \begin{equation*} \frac{\partial^2\phi}{\partial x^2}= \frac{1}{c^2}\,\frac{\partial^2\phi}{\partial t^2}, \end{equation*} and we saw that it described the propagation of waves in the $x$-direction at the speed $c$. Equation (18.26) is the corresponding wave equation for three dimensions. So in regions where there are no longer any charges and currents, the solution of these equations is not that $\phi$ and $\FLPA$ are zero. (Although that is indeed one possible solution.) There are solutions in which there is some set of $\phi$ and $\FLPA$ which are changing in time but always moving out at the speed $c$. The fields travel onward through free space, as in our example at the beginning of the chapter.
With Maxwell’s new term in Eq. IV, we have been able to write the field equations in terms of $\FLPA$ and $\phi$ in a form that is simple and that makes immediately apparent that there are electromagnetic waves. For many practical purposes, it will still be convenient to use the original equations in terms of $\FLPE$ and $\FLPB$. But they are on the other side of the mountain we have already climbed. Now we are ready to cross over to the other side of the peak. Things will look different—we are ready for some new and beautiful views.
- Well, not quite. They can be “absorbed” if they get to a region where there are charges. By which we mean that other fields can be produced somewhere which superpose on these fields and “cancel” them by destructive interference (see Chapter 31, Vol. I). ↩
-
Choosing the $\FLPdiv{\FLPA}$ is called “choosing a
gauge.” Changing $\FLPA$ by adding $\FLPgrad{\psi}$, is called a
“gauge transformation.” Equation (18.23) is called “the Lorenz gauge.”
2025.1.4: $A$는 $CP^2$의 고차원에 존재하는 긴데, 거기에 $\FLPgrad{\psi}$만큼의 자유가 있다는 거 ..... 자유의지
↩
뚱딴지 해설
4.23: $\FLPdiv{\FLPA}=\FLPdiv{\FLPA'}$ => $\FLPdiv({\FLPA}-{\FLPA'})=0$ => $A-A'= \FLPcurl{\text{something}}$